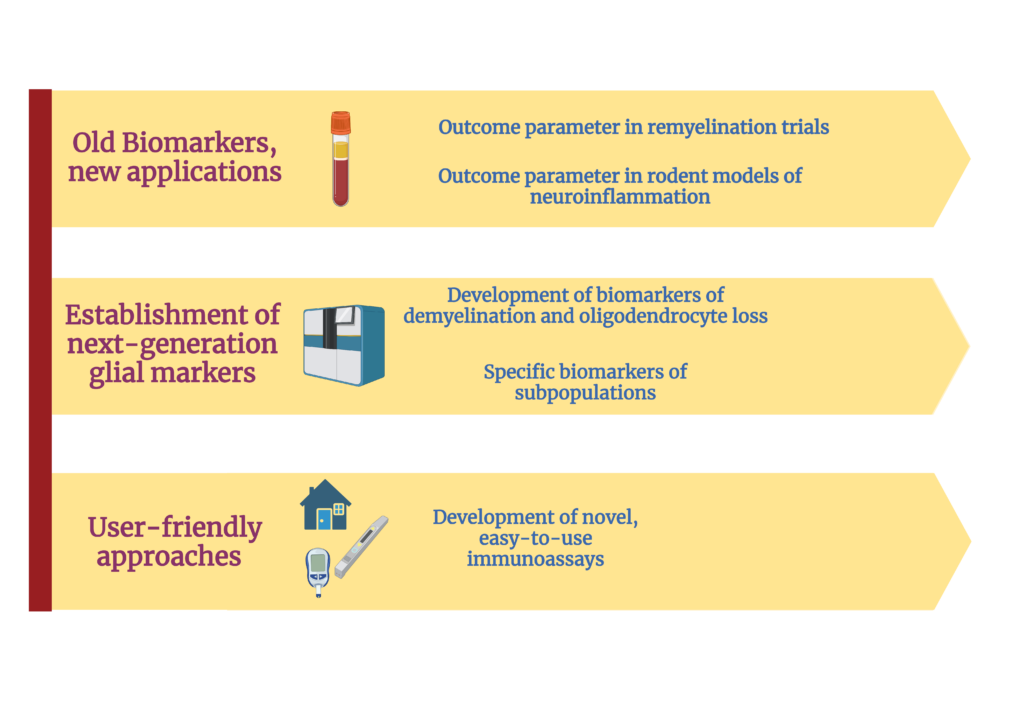
Brain imaging including magnetic resonance imaging (MRI) has helped revolutionize our understanding of structural injury in neurological disease including the field of MS research. Although brain imaging methods with some specificity for type of injury have been developed, methods for tracking the type of pathology and the specific cell types that manifest injury has been limited. We aim to use biomarkers derived from body fluids (including plasma, serum and CSF) to help monitor neurological diseases especially degenerative, progressive diseases of the central nervous system. Using an understanding of the biology of MS as well as other neurodegenerative diseases of the CNS we aim to select and identify promising candidate biomarkers for detection and assay optimization. We then aim to refine methods for the detection of possible CNS (and PNS) specific proteins and peptides including developing new tools for highly-quantifiable, highly-sensitive quantification of such proteins in blood for ready assessment and monitoring. Furthermore, we also aim to biologically validate these potential biomarkers using pre-clinical tools to document the source of these proteins and determine how such proteins and peptides get into the various compartments (blood, CSF, other body fluids) where they can be detected. Ultimately we aim to use these approaches to improve our capacity to study CNS injury in both animal models and human diseases dynamically and to try and understand the interplay between various pathological processes that produce neurological dysfunction.
This improved understanding will then be used to employ these improved biomarkers in ongoing and future clinical trials monitoring neuroprotective and neurorestorative therapies. The central aim of our projects is help monitor, understand and prevent irreversible damage to nerve cells and the nervous system.
In the context of MS: the ability to untangle the complexity of the various mechanisms that contribute to injury and to measure the effect of each of them independently is very limited. Therefore, our work focuses on the development, validation, and utilization of novel highly-sensitive technologies and state-of-the-art protein measurement and biomarker discovery tools to determine the protein signature in people with MS (pwMS) to:
- Understand the mechanisms that ultimately cause neurodegeneration and irreversible disability.
- Define the causes, and characterize the consequences of remyelination failure
- Develop novel tools that allow for the wide-spectrum application of biomarkers in different clincal and research settings
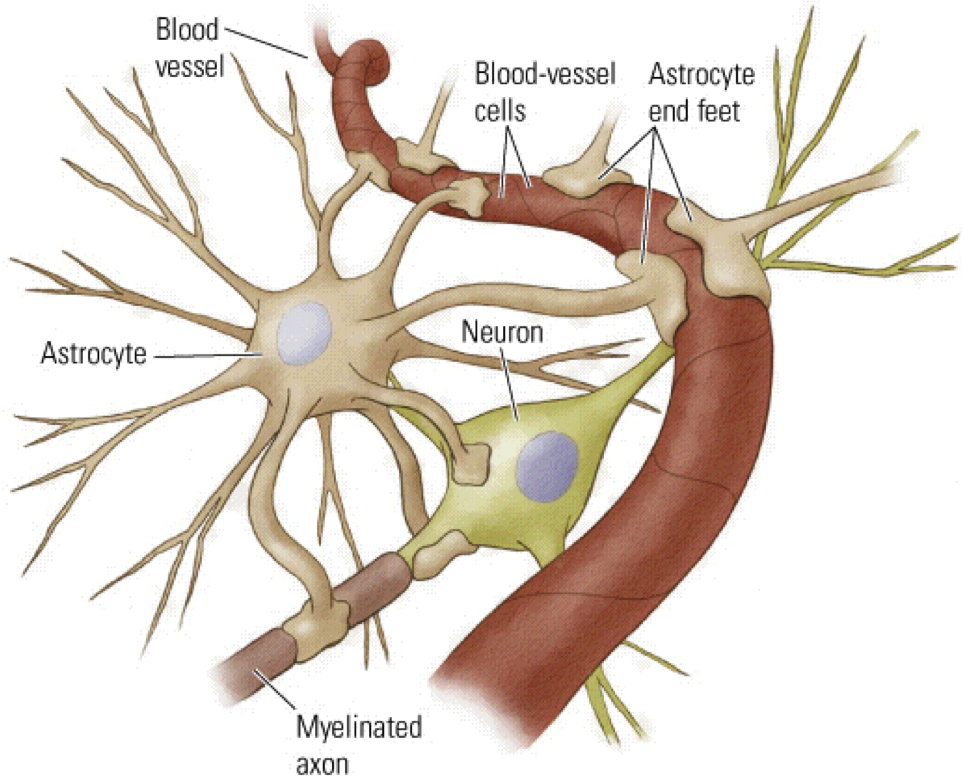
Here are some reasons why astrocyte biomarkers are used in MS research:
- Disease Pathology: Astrocytes are actively involved in the immune response and tissue repair processes in MS. They become activated in response to inflammation and release various molecules that can contribute to neuroprotection or damage. By studying astrocyte biomarkers, researchers aim to understand the specific functions and mechanisms astrocytes contribute to MS pathology.
- Neuroinflammation and Disease Activity: Astrocytes play a role in neuroinflammation, an essential process in MS. They can release proinflammatory molecules and contribute to forming the blood-brain barrier (BBB) breakdown. Astrocyte biomarkers, such as glial fibrillary acidic protein (GFAP) and S100B protein, are studied to assess disease activity and neuroinflammatory processes in MS.
- Disease Progression and Neurodegeneration: In MS, neurodegeneration occurs gradually and contributes to the development of disability over time. Astrocytes are involved in supporting neuronal health and synapse function. Dysfunction of astrocytes can lead to neurodegenerative processes. By identifying astrocyte biomarkers, researchers aim to understand the contribution of astrocyte dysfunction to disease progression and neurodegeneration in MS.
- Treatment Responses: Astrocytes may be involved in responding to MS treatments, including disease-modifying therapies (DMTs). Biomarkers associated with astrocyte activity can provide insights into treatment effects on astrocyte function, inflammation, and neuroprotection. Monitoring astrocyte biomarkers may help evaluate treatment responses and assess the efficacy of different therapeutic approaches in MS.
- Therapeutic Targets: Identifying astrocyte-specific biomarkers can aid in the development of new therapeutic targets for MS. Understanding the specific molecular pathways and signaling mechanisms in astrocytes can potentially lead to the development of novel treatment strategies aimed at modulating astrocyte function and neuroinflammation.
It’s important to note that astrocyte biomarker research is still ongoing, and further studies are needed to validate and establish their clinical utility in MS diagnosis, prognosis, and treatment. However, the investigation of astrocyte biomarkers holds promise in advancing our understanding of MS pathophysiology and improving therapeutic approaches in the future.